The Neuse River waterdog lives a sluggish existence, as if burdened by an invisible weight. This mottled brown salamander, about as long as a human hand, rarely strays far from its concealed burrows beneath rocks or logs in the rivers of North Carolina. It “hunts” by sitting still in the riverbed, waiting for an insect to swim by, then lurches forward to swallow the object—a mindless reflex. It spends its entire life confined to water, an overgrown larva that never completes metamorphosis, with flaccid legs too small for its body, toes that haven’t finished sprouting, a missing upper jawbone and puffy larval gills bulging from its neck.
View the animal up close, and another oddity becomes apparent: its cells are up to 300 times larger than those of a lizard, bird or mammal. You can see, with a simple magnifying glass, individual blood cells zipping through the capillaries in its transparent gills.
The Neuse River waterdog, Necturus lewisi, and other salamanders represent a long-standing conundrum scientists are only now starting to understand. The animal’s strange traits stem from a hidden burden: Each of its cells is bloated with 38 times more DNA than a human cell. The waterdog has the largest genome of any four-footed beast on Earth. The only comparable animals of any kind are lungfish, which also have sluggardly tendencies.
Most mammal, bird, reptile and fish genomes fall within a narrow range of half a billion to six billion chemical building blocks, or base pairs, of DNA. The base pairs form genes—links in a long chain that constitute an animal’s genome. But salamander genomes range wildly, from 10 billion to 120 billion base pairs (10 to 120 gigabases). Salamanders don’t have more genes than other animals; instead their genomes are cluttered with segments of parasitic DNA that have multiplied out of control. Everything about their lives is dominated by their massive genome, which has pushed them into the extreme slow lane of existence. They slog through life with underdeveloped bodies, simplified brains and hearts as flimsy as paper bags, sometimes for 100 years.
In exchange for this burden, salamanders may have gained at least one amazing ability: regeneration. They can regrow not only limbs but also up to a quarter of their brain if it is cut out—handy for survival.
Salamanders owe their weird traits to their DNA, but not in the way you’d expect. DNA is often called the blueprint of life. It contains the precise information that determines the structure and function of every cell in every species. But the latest discoveries about salamanders upend this long-held notion of a fine-tuned genome. They reveal that DNA also shapes its owner in ways that have nothing to do with its informational content. DNA can distort bodies and organs like a funhouse mirror; a species can tolerate only so much DNA before experiencing those side effects. We humans, in fact, may be close to our limit: make our genome any larger, and it could compromise our species’ greatest asset, our intelligence.
As for salamanders, one has to wonder why their burden hasn’t dragged them down to extinction. Their very perseverance suggests that our idea of evolution, particularly “survival of the fittest,” has a serious moralistic bias: Work hard, young species, hone your body and brain for high performance, and someday you will succeed. But salamanders owe their success to lying around. They have found a way to cheat the system.
Inflated Genomes
The mystery of gigantic genomes began during a pivotal moment decades ago, when biologists had just identified DNA as the hereditary molecule of life. A genome, unique to every species, contains thousands of genes, composed of DNA, that instruct cells to make proteins and other molecules that make an organism what it is. Researchers initially assumed that advanced species with complex bodies, such as primates and humans, would have more genes and therefore larger genomes.
But Alfred Mirsky and Hans Ris of the Rockefeller Institute for Medical Research overturned this notion in 1951. They measured the amount of DNA in individual cells from several dozen animal species. To their surprise, the African lungfish and a giant salamander from the southeastern U.S., called amphiuma, had dozens of times more DNA per cell than humans, rats, birds or reptiles did. As scientists measured more species, it became clear that salamanders and lungfish were outliers.
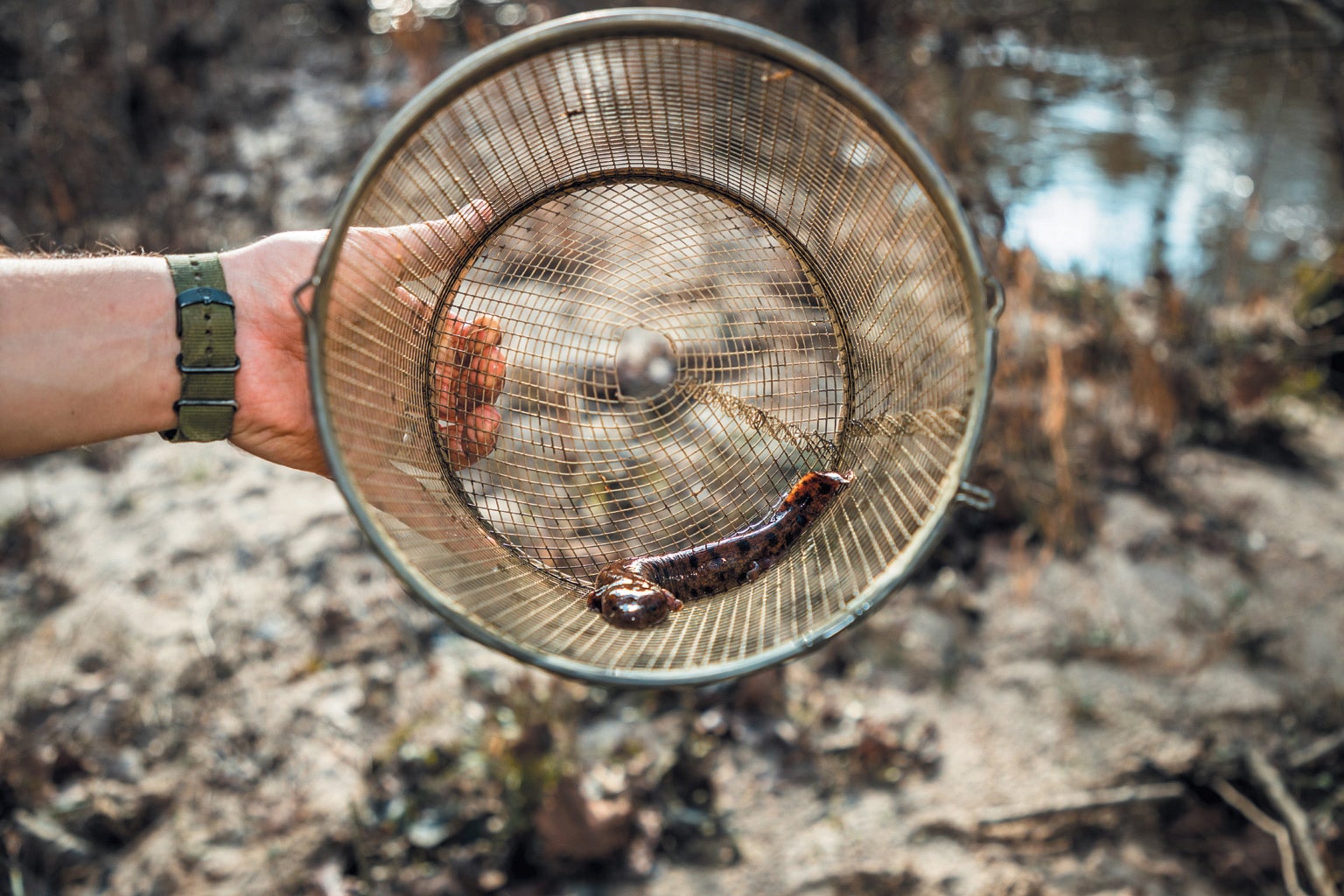
Over the next two decades researchers got a closer view of gigantic genomes. Shigeki Mizuno and Herbert MacGregor of the University of Leicester in England studied a handful of North American salamander species called plethodontids. The species looked nearly identical, yet their genomes ranged from 18 to 55 gigabases—about five to 16 times the human genome, which has 3.06 gigabases.
In all the species, the DNA chain was wound into sausage-shaped structures called chromosomes. But in species with bigger genomes, the chromosome shape looked enlarged, like an overinflated sausage balloon. Extra DNA seemed to be sprinkled throughout the chromosomes’ length.
Mizuno and MacGregor had no idea what that extra material was. But during the 1980s scientists found that cells in other species, from flies to humans, harbored “parasitic” DNA—short DNA segments, called transposons, which vaguely resemble viruses. Transposons contain several genes that allow the parasite to make copies of itself, which then insert themselves, sometimes randomly, into other parts of a cell’s genome.
The exploration of gigantic genomes went slowly for several decades. Scientists labored to fully sequence the genomes of fruit flies, worms and humans, but most avoided salamanders, whose sheer volume of DNA would have been a nightmare to handle. Then in 2011 Rachel Mueller, an evolutionary biologist at Colorado State University, took a major step forward.
Mueller and her colleagues used high-throughput sequencing to analyze hundreds of thousands of random DNA snippets from six species of plethodontid salamanders, as well as another species called hellbender. The results confirmed what people had suspected: salamander genomes were overinflated with transposons. Many of the same transposons were present in both the plethodontids and hellbender, which suggested that the parasites had first multiplied out of control in the ancestor of all living salamanders, more than 200 million years ago.
The mystery of why that explosion happened has intrigued Mueller ever since. “It wasn’t like one [transposon] went bananas,” she says. “It was a global change in how those [transposon] sequences were permitted to inhabit the genome,” allowing dozens of them to multiply simultaneously.
Although Mueller hasn’t determined why, she has solved another puzzle: Even when transposons do proliferate in a host’s genome, they are usually deleted over time through random mutations. This pruning happens constantly in every species. But in two studies, Mueller estimated that salamanders clear out their inserted transposons several times more slowly than zebra fish or humans. This pace shifts the balance toward transposons accumulating rather than remaining constant, leaving the salamander genomes increasingly bloated over time.
That extra DNA has profoundly altered salamanders’ bodies, brains and hearts. In species with the very largest genomes, the anatomic distortions are obvious at a glance.
Embryonic Brains
Gigantic genomes often turn salamanders into overgrown babies. Of the 766 known species, more than 39 have lost the ability to metamorphose from aquatic larvae into landlubbing adults. (Another 39 metamorphose only occasionally.) These species tend to have larger genomes than others that do metamorphose. Like the Neuse River waterdog, they spend their entire lives confined to water with larval gills and weakling limbs.
Many of them are also missing toes because their limbs never finish developing. The waterdog has only four toes on its rear feet (most salamanders have five). Species of amphiuma have three, two or even just one toe per foot. And species in the siren family, which inhabit the southeastern U.S., have no rear legs at all.
Even land-dwelling salamanders with adult-looking bodies often have babylike traits, such as unfused skull bones or foot skeletons that haven’t hardened into bone. A series of discoveries between 1988 and 1997 showed that many of these species even have larvalike brains.
The revelations began when David Wake, a prominent salamander biologist at the University of California, Berkeley, teamed up with Gerhard Roth, then a Ph.D. student at the University of Bremen in Germany. They decided to compare the brain structures of several dozen species of frogs and land-dwelling plethodontid salamanders.
Wake removed the critters’ brains and soaked them in cedar wood oil, which turned them transparent for viewing under a microscope. As he and Roth examined the brains, they realized that most had simpler structures than those of frogs, which are also amphibians and therefore close relatives. The salamander nerve cells looked “embryonic,” according to Wake: larger, rounder and less differentiated into specialized cell types.
This simplification was especially dramatic in the visual system. The salamanders had no more than 75,000 neural fibers in their optic nerves (which carry signals from the eyes to the brain); frogs had up to 470,000 fibers. In salamanders, far fewer of these nerve fibers were coated with myelin sheaths, which allow signals to reach the brain more quickly. And in the tectum, a brain region that processes images from the optic nerve, the neurons of salamanders were often strewn chaotically about—a trait seen in embryonic or larval brains—whereas in frogs, the neurons were nicely layered. Wake and Roth went on to show that salamanders with larger genomes generally have simpler visual systems.
In all of this, Roth was struck by one overarching pattern: the features missing in salamander brains were those that arise late in development. It seemed as if the critters’ brains had run out of time to finish maturing. This stipulation made a lot of sense because another scientist had just demonstrated a link between salamanders’ large genomes and their slow development.
Stanley Sessions, a former student of Wake’s (like several of the experts in this story), was studying salamanders’ special talent for regenerating severed limbs. Sessions, now a professor emeritus at Hartwick College, amputated the right rear legs from 27 species of plethodontid salamanders and measured how quickly they grew back. The animals’ genomes varied from 13 to 74 gigabases (four to 24 times the human genome). Sure enough, Sessions found that animals with larger genomes regenerated more slowly. Their immature cells took longer to differentiate into specialized tissues such as muscle or bone.
The studies by Wake, Roth and Sessions also provided a rationale for understanding why salamanders with some of the largest genomes of all had lost toes, hind legs and even their ability to undergo metamorphosis. Their hefty genomes had slowed and truncated many aspects of development. People assumed that this depressed development stemmed from the simple fact that large genomes take longer to copy, so cells divide more slowly. But in 2018 a new milestone in genomics provided a critical insight.
Researchers published the first complete salamander genome, for the Mexican axolotl. This beast can grow almost as long as a person’s forearm. It has pencil legs, fluffy gills and other larval traits but has a genome of “only” 32 gigabases, compared with the waterdog’s 118 gigabases. This study showed that the animal’s transposons aren’t just scattered among its genes; they are also abundant within genes, in regions called introns.
This minor detail has enormous implications. When a gene is turned on, its entire length of DNA, including introns, must be copied into a chain of RNA. The introns must then be clipped out before the RNA chain can be used as a template to make proteins that will guide a cell’s development. Axolotl introns are up to 13 times longer than human introns, because they are crowded with transposons. Therefore, the RNA chains take longer to build. The instructions for how cells should specialize take longer to exert their effects—so long, Sessions says, that salamanders “never quite grow up.”
Slow development is only part of how giant genomes distort bodies. Massive genomes have another major impact. Scientists accidentally noticed it more than 150 years ago, but its importance is just now being recognized.
Hearts Like Paper Bags
During the early 1800s a British army surgeon named George Gulliver pursued a pet interest as he traveled the world. At each destination he collected blood from local species, viewed the samples under a microscope and measured the red blood cells. He surveyed Mexican deer, American crocodiles, Indian pythons, spiny dogfish, electric eels, armadillos and hundreds of other animals. Gulliver found the largest cells by far in the three-toed amphiuma, whose vestigial legs are so tiny it resembles an eel. Its red blood cells occupied 300 times more volume than those of humans. Salamanders and a lungfish were close behind amphiuma, with the next-largest cells.
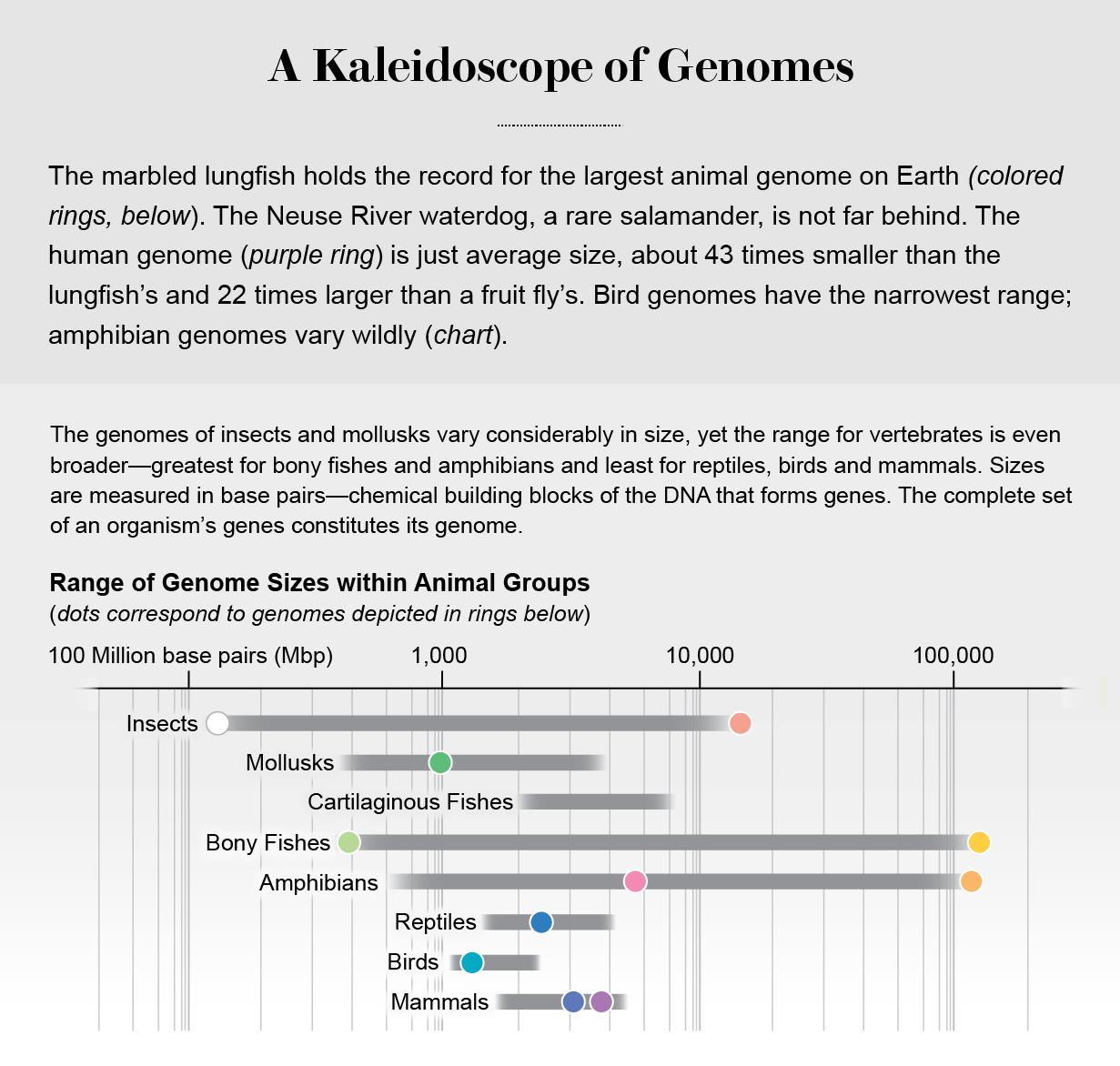
We now know that cell size and genome size go hand in hand: the more DNA, the larger the cell. Large cells have significant effects on an animal’s structure. Some salamanders have responded by simply growing really big bodies. The Chinese giant salamander can be 1.8 meters long. Amphiuma species can reach 1.1 meters. The Neuse River waterdog can approach 28 centimeters, which is still twice as long as most other salamanders.
Large cellular building blocks also result in simpler bodies. Imagine that you’re building two identical toy cars—one with small Lego blocks, the other with big Duplo blocks. If the cars are the same size, the one made with bigger blocks will have a simpler, more chunked-up design. That’s how salamander bodies appear.
James Hanken, now at Harvard University, discovered the classic case of this in the 1980s. Hanken was studying wrist “bones” (actually made of cartilage that never finishes hardening) in the world’s smallest salamanders. These species of the genus Thorius inhabit nooks in Mexico’s montane forests. Some are small enough to sit on the face of a nickel. Dozens of related species have the same eight wrist bones, despite evolving separately for millions of years. But Hanken found that in Thorius species, some of the eight ancestral bones had merged. More surprisingly, the arrangement of bones varied within a single species. Some animals had as few as four wrist bones, others as many as seven. Some even had different bone patterns in their right and left wrists.
That kind of variability was “exceptional,” Hanken says. He thinks that because Thorius has a small body and big cells, there literally aren’t enough cells to go around when wrist bones form in the embryo.
Mueller and her Ph.D. student Michael Itgen were fascinated by Hanken’s conclusion that larger cells lead to simplified bodies. But they wondered whether it actually mattered to these animals. In 2019 they started an ambitious project to understand how differences in cell size influence the structure of the heart; they looked at nine species of plethodontid salamanders with genomes ranging from 29 to 67 gigabases.
Plethodontids are lungless; they breathe through their skin. They have only one heart ventricle (rather than two, as mammals do). As Itgen examined the plethodontids’ ventricles under a microscope, he was astonished at how different they were. Animals with the smallest genomes had muscular, thick-walled ventricles, with only a small space for blood in the center. As the animals’ genomes escalated, their ventricles became increasingly hollowed out, with a larger blood cavity surrounded by ever thinner muscle walls. In the species with the largest genome, the ventricle resembled an empty bag made of a flimsy film of muscle, as little as one cell thick.
Seeing that hollow heart was a revelation. “I can’t even imagine how that thing functionally works,” says Itgen, who, along with Mueller, submitted his results to the journal Evolution in late 2021.
Itgen isn’t sure why larger genomes lead to hollower hearts. He speculates that the ventricles of larger-genome species may need more space to accommodate larger blood cells, which can change blood’s viscosity. Or, he says, the hollow hearts might have less muscle because the cells can’t divide quickly enough during development.
Either way, this shoddy construction comes at a heavy price. Adam Chicco, who studies cardiac physiology at Colorado State University, sees parallels between these thin-bag ventricles and what he has observed in humans with severe heart failure: fewer muscle cells, stretched ever thinner, less and less able to pump blood.
The salamanders would be on death’s door if they were human. “Everything about having a large genome is costly,” Wake told me in 2020. Yet salamanders have survived for 200 million years. “So there must be some benefit,” he said. The hunt for those benefits has led to some heretical surprises, potentially turning our understanding of evolution on its head.
Profound Distortions
Wake spoke with me twice in 2020; he died in April 2021. But by then, he and Sessions had finally reached an insight that had eluded them for decades: a theory of how salamanders and lungfish might benefit from outsized genomes. The theory germinated from an audacious experiment.
Sessions and his undergraduate student, Yuri Mataev, had anesthetized several eastern newts, peeled back the thin flaps of their skulls and removed nearly a quarter of each animal’s brain—a region involved in smelling. It’s one thing for a salamander to regenerate a severed leg; Sessions wanted to test the limits of this ability. Sure enough, “within six weeks they were regenerating their brains,” Sessions says.
The experiment showed that salamanders could regrow body parts they don’t normally lose in nature. That concept was at odds with a basic evolutionary principle—that abilities arise in response to environmental stressors. Perhaps, Sessions suspected, regeneration had evolved only partly in response to such stressors, and a giant genome had enhanced the tendency as an ultimately beneficial side effect.
Sessions now thinks that slow development, caused by transposons located within introns, might leave adult salamanders full of immature cells that can still differentiate into new tissues. “Salamanders are basically walking bags of stem cells,” he says. This theory, which he put forth with Wake, was published in June 2021, shortly after Wake died.
The idea has “some plausibility,” says Jeramiah Smith, who studies the axolotl genome at the University of Kentucky. He cautions that the picture may not be so simple; life has many ways of slowing down development when it is advantageous to do so. But with transposons so abundant in the salamander genome, it makes sense that they would play a role. “Evolution works with the material it has,” Smith says.
The theory, if true, could have major repercussions. Scientists have spent decades studying salamander regeneration, in hopes of finding ways to regrow human tissues. But if regeneration requires having a multitude of genes with long introns, that could make the goal more challenging.
On a more profound level, Wake and Sessions’s theory reflects the great depth to which genetic parasites have reprogrammed the very biology of salamanders. Many long-lived species such as humans keep their remaining stem cells muzzled once development is complete, an evolutionary trade-off that reduces the ever present risk of runaway cell division, which can cause cancer. Salamanders’ stem cells are more numerous and far less constrained.
Wake and Sessions’s theory may not fully explain why salamanders can tolerate huge genomes. Although it is handy to be able to regrow appendages that are lost on rare occasions, salamanders still have to survive day after day with those bizarre distortions of their hearts, brains and bodies. This paradox points to a surprising possibility, which emerged during a conversation between Mueller, Itgen and Hanken in mid-2021.
The trio were on a Zoom call, discussing how the hollowed-out hearts might affect the survival of salamanders. “I’ll take the extreme position,” Hanken said. Maybe the hollow hearts “are not having any impact” at all.
Strange as it might seem, that suggestion made sense to Mueller and Itgen. Salamanders grow and move slowly. They have by far the lowest metabolic rates and oxygen needs of any vertebrates. The plethodontids that Itgen and Mueller study don’t even have lungs. Maybe salamanders tolerate hollow ventricles, Itgen said, “because the functional requirements on the heart are so low.”
Indeed, when Sessions ran his regeneration experiments, he also removed up to half of the lone ventricle in a dozen eastern newts. The blood gushed out, and the hearts stopped beating, yet the animals survived and grew new ventricles—suggesting that they may not need their hearts as much as mammals do.
Salamanders don’t seem to pay a price for their odd skeletons, either. Hanken thinks Thorius tolerates slipshod wrist bones because the animals’ bodies are so small that the forces on their joints are minuscule. And Thorius doesn’t need the fine-tuned limbs of a cheetah, because it doesn’t chase its prey. It simply sits and waits for an insect to happen by.
Roth adds that if salamanders are just waiting for prey, they can simplify their entire vision system. The most extreme examples are the bolitoglossine salamanders of Europe and the Americas. These species include the largest genomes of any land-dwelling animals, up to 83 gigabases (24 times the human genome). They also happen to have the most stripped-down brains that Roth and Wake have ever seen in a salamander. They have lost 50 to 90 percent of their visual neurons to simplification, leaving them unable to distinguish between an insect crawling past them and a shiny metal pellet rolling by. What bolitoglossines do have, however, is one of the fastest tongues on Earth—“like walking around with a cocked gun,” Wake said—able to zap an insect within a few milliseconds.
If you have that kind of tongue, if you don’t need to see very well, if you can sit still for long periods, all that takes a lot of pressure off the body. You can have a simplified brain, a hollow heart and weird wrist bones, “and it doesn’t matter,” Mueller says. “It’s pretty profound.”
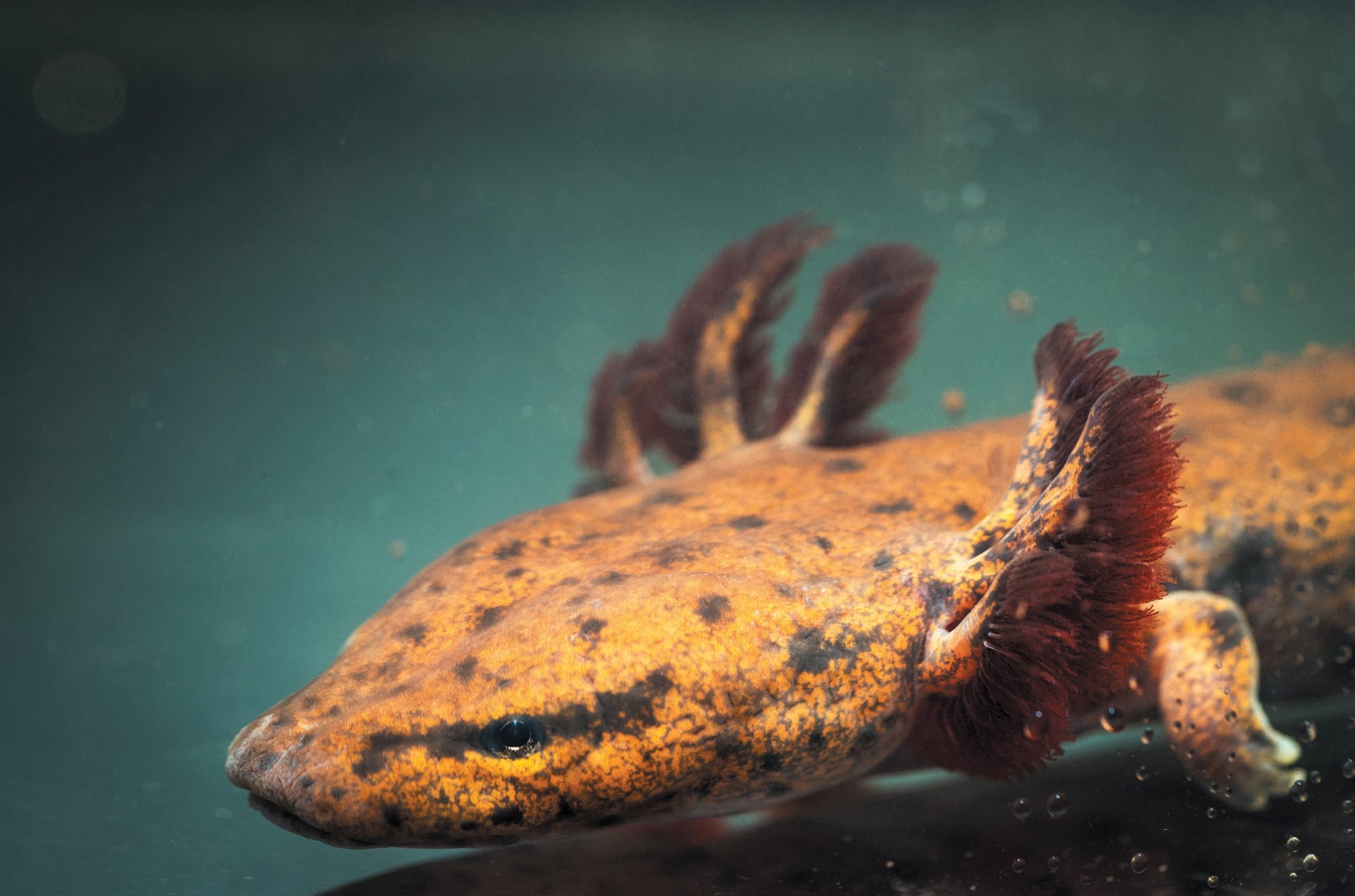
Cruel Irony
Ever since it became clear that salamanders and lungfish have far more DNA than humans, scientists have debated what purpose this extra DNA might serve. Initially some of them argued that DNA, in addition to its informational content, serves as a scaffold that determines the size of a cell’s nucleus. That idea has fallen by the wayside. The latest view is more nuanced.
Transposons are indeed junk DNA, says Ting Wang, a genomic scientist who studies transposons at the Washington University School of Medicine in St. Louis. But this junk, scattered across the genome, becomes fodder for evolution. Sometimes it takes on legitimate functions. Transposons that land close to a gene can cause the gene to turn on more strongly, for example. In 2021 Wang discovered a transposon that activates a critical gene in mouse embryos; delete that single transposon, and many of the embryos die. Transposons also play structural roles, partitioning our genome into functional sections. “You can’t separate them from us anymore,” Wang says. “They’re part of us.”
And yet they can betray us. When Wang’s team analyzed nearly 8,000 human tumors in 2019, the researchers found that in half, transposons were turning on key oncogenes that were driving the cancer’s explosive growth.
All of this suggests that although transposons are sometimes coopted by the host, they have no inherent purpose. “Not everything is adaptive,” says T. Ryan Gregory, a biologist who studies genome size at the University of Guelph in Ontario. DNA exists for its own sake. It doesn’t just evolve to maximize the survival of its host; it also evolves to maximize itself—the host be damned.
As the host struggles to maintain its niche in the world, an equally dramatic struggle plays out in its cells. Transposons compete to populate the genomic landscape and evade predation by cell defenses. “We’re starting to think about the genome as an ecological community and the transposable elements as species,” Mueller says.
The propensity of transposons to multiply means that all genomes have a tendency to expand over time. Like stuff in a garage, DNA will accumulate to fill whatever space is available. It is the pressure of natural selection, penalizing the host when its genome gets too big, that maintains the genome at a certain size in most species. There are patterns to that size, Gregory says. The DNA load that a species can tolerate depends on its speed of development, its metabolic rate and the way it lives.
Birds, with their fast metabolism and energy-demanding flight, simply can’t manage a lot of bulky DNA. Their genomes are smaller than those of most mammals, ranging from 0.89 to 2.11 gigabases—fewer than the 3.06 gigabases for humans. Among mammals, 19 of the 20 smallest genomes belong to bats, which face challenges similar to birds’.
We humans fall in the middle of the mammal pack, which probably reflects several competing factors. We develop slowly, taking almost 20 years to reach adulthood, which implies that we should have significant capacity for carting around extra DNA. But the size of our genome may sit on a razor’s edge, kept in check by another critical factor: the brainpower that we depend on for survival. Suzana Herculano-Houzel, a brain scientist at Vanderbilt University, thinks humans and other primates owe their outsize intelligence to the fact that their nerve cells are relatively small, allowing us to cram more of them into our cerebral cortex. If her theory is correct, then a larger human genome might leave us with fewer brain cells—and less smarts.
Frogs and toads, close relatives to salamanders, often have relatively large genomes, ranging up to 13.1 gigabases of DNA. But the ornate burrowing frog, whose genome was published in 2021, has only 1.06 gigabases, similar to a hummingbird’s. It inhabits the Australian desert, laying eggs in puddles that form after infrequent rains. The tadpoles have only a few days to sprout legs before their aquatic nurseries evaporate. They simply can’t afford to hoard genomic junk. Even among plants, the fast-spreading weeds that take over vacant lots—thistle, dandelions, and the like—often have smaller genomes than the slower-growing species that they smother.
In contrast to lean-and-mean species, the salamander probably evolved its bloated genome gradually. Gregory and Mueller think that 200 million years ago, the ancestor of all salamanders probably occupied the slow lane of life, with low energy needs and sluggish development. As a result, it suffered no immediate harm as transposons accumulated in its genome. As salamander genomes expanded, they pushed the critters further into niches where the slow-and-frugal strategy paid off.
In a 2020 paper, Gregory suggests that this process eventually hit a tipping point: transposons changed from being mere inhabitants of the genomic landscape to being full-blown ecosystem engineers. When a transposon inserts a new copy of itself, there is always a risk that it will disrupt a gene and harm the host—which is bad if you’re a parasite because you depend on the host for survival. A newly inserted transposon won’t be passed on to the next generation if it causes its host to be sterile, for example. But as transposons multiplied, their very presence provided more “habitat” in the genome where new transposons could insert themselves without hitting genes. “There is a feedback loop,” Gregory says. “The more transposons you have, the more safe places you have to insert them.”
And so it was that the Neuse River waterdog eventually found itself lugging around 118 gigabases of DNA. Its sister species, the dwarf waterdog (Necturus punctatus), is right behind, with 117 gigabases.
It’s easy to look at the Neuse River waterdog and feel a pang of pity. Its slow development not only leaves it unable to metamorphose but may also prevent adult salamanders from regenerating limbs, a cruel irony. Unable to traverse dry land, the waterdog remains isolated in two small river systems in North Carolina. Agriculture and development have led to worsening water quality. In June 2021 the U.S. government listed the waterdog, whose population is falling, as “threatened.” Although salamanders have survived as a group for 200 million years, it is tempting to think that this one species’ ginormous genome has pushed it toward extinction.
Sessions isn’t so sure. These bloated beasts have demonstrated, time and again, that when it comes to survival of the fittest, our notion of “fitness” is biased toward strength and agility. Genomic parasites have slowed the waterdog’s development, swelled its cells and distorted its anatomy. This odd circumstance has pushed the animal onto a bizarre evolutionary side track that redefines fitness in such a way that hearts and complex brains are reduced to an afterthought. Yet somehow the animal’s lineage persists, even as fires, floods and asteroids obliterate other species—furry, feathered and scaled—that seem more fit.
“Salamanders,” Sessions says, “are tough survivors.”