For astronomers, it’s a magical moment: you’re staring at a monitor, and a blurry image of a cosmological object sharpens up, revealing new details. We call this “closing the loop,” a reference to the adaptive optics loop, a tool that enables telescopes to correct for haziness caused by turbulence in the atmosphere. Adaptive optics essentially untwinkles the stars, canceling out the air between us and space to turn a fuzzy image crisp.
One night last year our team at the Australian National University was closing the loop on a new imaging system made to resolve the details of space debris. Sitting in the control room of our observatory on Mount Stromlo, overlooking the capital city of Canberra, we selected a weather satellite for this first test. It was an easy target: its large body and solar panels are unmistakable, offering a good way to test the performance of our system.
For some of us, this was the first time we had used a telescope to observe something that was not a star, galaxy or other cosmic phenomenon. This satellite represents one of the thousands of human-made objects that circle our planet, a swarm of spacecraft—some active, most not—that pose a growing risk of overcrowding near-Earth orbits. Our test was part of an effort to build systems to tackle the problem of space debris and preserve these orbital passages for future use. It is one of several new ways that we are using adaptive optics, which has traditionally been used for astronomical observations, to accomplish different goals. After more than three decades of perfecting this technology, astronomers have realized that they can apply their expertise to any application that requires sending or receiving photons of light between space and the ground.
The Fight against the Atmosphere
The layer of gas between Earth and the rest of the cosmos keeps us alive, but it also constantly changes the path of any photon of light that travels through it. The culprit is atmospheric turbulence caused by the mixing of air of different temperatures. Light bends, or refracts, when it travels through different mediums, which is why a straw in a glass of water looks like it leans at a different angle under the water than above it—when the light bouncing off the straw moves from water into air, it changes course. The same thing happens when light travels through air of different temperatures. When light passes from warm air to cool air, it slows down and its path changes.
This effect is why stars twinkle and why astronomers have such a hard time taking precise images of the sky. We quantify the impact of atmospheric turbulence with “the seeing,” a parameter that describes the angular size of the blurred spot of a star as seen through a ground-based telescope. The more turbulent the atmosphere, the worse the seeing. At a good site, on a high mountain with low turbulence, the seeing is typically between 0.5 and one arcsecond, meaning that any telescope will be limited to this range of resolution. The problem is that modern telescopes are capable of resolution significantly better than that. From a purely optical point of view, the resolution of a telescope is dictated by the “diffraction limit,” which is proportional to the wavelength of the light that is collected and inversely proportional to the diameter of the telescope collecting that light. The wavelengths we observe depend only on the chemical composition of our celestial targets, so those cannot be changed. The only way to build telescopes that can resolve smaller and smaller objects is therefore to increase their diameter. A telescope with a two-meter diameter mirror can, for example, resolve objects that are 0.05 arcsecond in optical wavelengths (the equivalent of resolving a large coin 100 kilometers away). But even a very good site with low seeing will degrade this resolution by a factor of 10.
It is thus easy to see the attraction of putting telescopes in space, beyond the reach of the atmosphere. But there are still very good reasons to build telescopes on the ground. Space telescopes cannot be too large, because rockets can carry only so much weight. It is also difficult to send humans into space to service and upgrade them. The largest space telescope currently under construction is the James Webb Space Telescope, and its primary mirror is 6.5 meters wide. On the ground, the largest telescope mirrors are more than 10 meters wide; now being built, the Extremely Large Telescope will have a primary mirror that extends 39 meters. Ground-based telescopes can also be upgraded throughout their lifetimes, always receiving the latest generation of instrumentation. But to use these telescopes to the fullest, we must actively remove the effects of the atmosphere.
The first adaptive optics concepts were proposed in the early 1950s and first used in the 1970s by the U.S. military, notably for satellite imaging from the ground. Astronomers had to wait until the 1990s to apply the technology in their observatories. Adaptive optics relies on three key components. The first is a wavefront sensor, a fast digital camera equipped with a set of optics to map out the distorted shape of the light waves heading toward the telescope. This sensor measures the distortion caused by the atmosphere in real time. Because measurements must keep up with fast changes in the atmosphere, it needs to make a new map several hundred to several thousand times per second. To get enough photons in such short exposures, the wavefront sensor requires a bright source of light above the atmosphere. The stars themselves are rarely bright enough for this purpose. But astronomers are a resourceful bunch—they simply create their own artificial stars by shining a laser upward.
This reference light source—the laser guide star—is the second key component of the adaptive optics system. Our atmosphere has a layer of sodium atoms that is a few kilometers thick and located at an altitude of 90 kilometers, well above the turbulence causing the distortions. Scientists can excite these sodium atoms using a specially tuned laser. The sodium atoms in the upper atmosphere absorb bright orange laser light (the same color emitted by the sodium street lamps in many cities) and then reemit it, producing a glowing artificial star. With the laser attached to the side of the telescope and tracking its movements, this artificial star is always visible to the wavefront sensor.
Now that we can continuously track the shape of the wavefront, we need to correct for its aberrations. This is the job of the third major component of the system: the deformable mirror. The mirror is made of a thin reflective membrane, under which sits a matrix of actuators, mechanisms that push and pull the membrane to shape the reflected light. Every time the wavefront sensor makes a measurement, it sends this information to the mirror, which deforms in a way that compensates for the distortions in the incoming light, effectively removing the aberrations caused by the atmosphere. The atmosphere changes so fast that these corrections must be made every millisecond or so. That is a major mechanical and computational challenge. The deformable mirror hardware must be capable of making thousands of motions every second, and it must be paired with a computer and wavefront sensor that can keep up with this speed. There are up to a few thousand actuators, each moving the deformable mirror surface by a few microns. Keeping up with this constant updating process in a self-correcting fashion is what we mean by “closing the loop.”
Although the technique is difficult and complex, by now astronomers have largely mastered adaptive optics, and all major optical observatories are fitted with these systems. There are even specialized versions used for different types of observations. “Classical” adaptive optics uses only one guide star and one deformable mirror, which enable atmospheric turbulence correction over a rather limited patch of sky. More complex systems such as Multi Conjugate Adaptive Optics use multiple guide stars and multiple deformable mirrors to probe and correct for a larger volume of atmospheric turbulence above the telescope. This approach opens up windows of atmospheric-free astronomical observations that are 10 to 20 times larger than what classical adaptive optics can achieve—but at a significantly higher price. In other situations—for example, when astronomers want to study an individual target, such as an exoplanet—the important factor is not field size but near-perfect image resolution. In this situation, an Extreme Adaptive Optics system uses faster and higher-resolution wavefront sensors and mirrors, usually coupled with a filter to block the light of the host star and enable imaging of the dim exoplanets orbiting it. We have now reached an age where it is not a stretch to expect any telescope to come with its own adaptive optics system, and we are beginning to expand the use of this technology beyond astronomy.
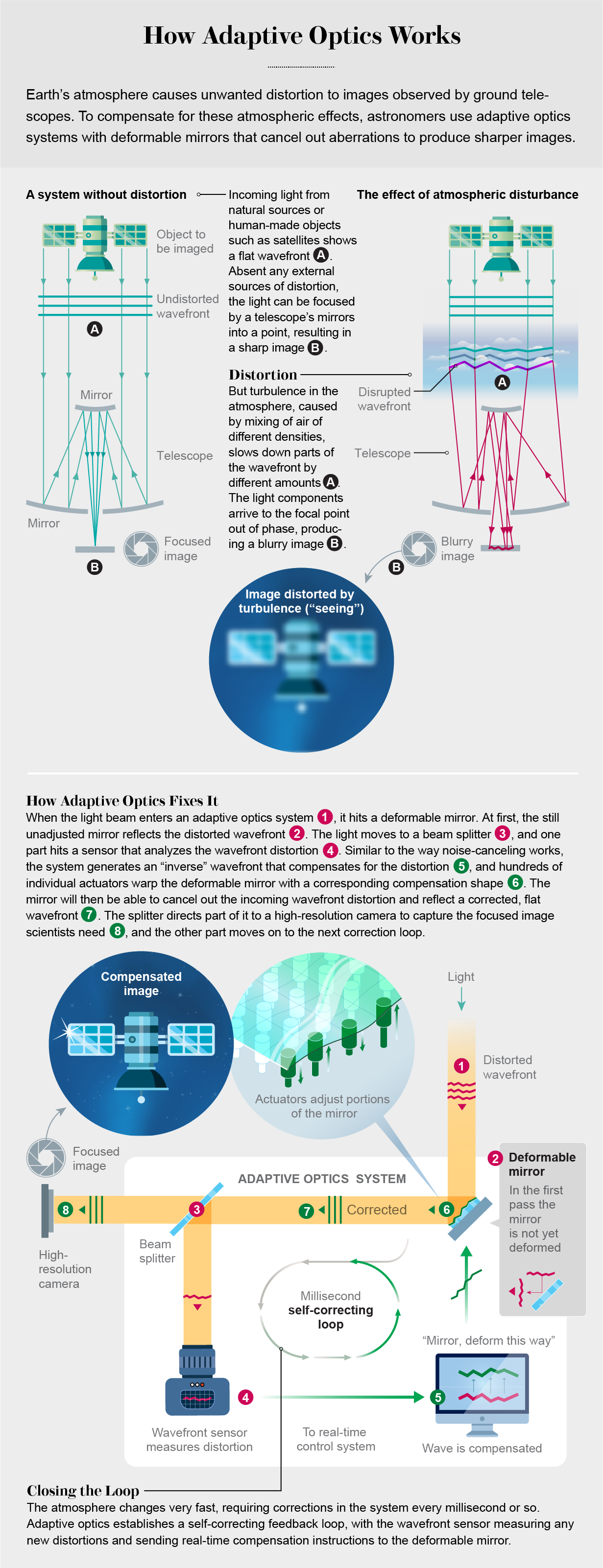
The Problem of Space Junk
Ironically one of these new applications helped to inspire the early development of adaptive optics: the observation of objects in close orbit around our planet. This research area, commonly called space situational awareness, includes the observation and study of human-made objects (satellites) as well as natural objects (meteoroids). A legitimate fear is that the ever increasing number of spacecraft being launched will also increase the number of collisions between them, resulting in even more debris. The worst-case scenario is that a cascading effect will ensue, rendering certain orbits completely unusable. This catastrophic, yet rather likely, possibility is called the Kessler syndrome, after Donald J. Kessler, the NASA scientist who predicted it as early as 1978.
About 34,000 human-made objects larger than 10 centimeters are now orbiting Earth; only about 10 percent are active satellites. Space junk is accumulating at the altitudes most heavily used for human activities in space, mainly in low-Earth orbit (some 300 to 2,000 kilometers above the ground) and geostationary orbit (around 36,000 kilometers). Although we can track the larger objects with radar, optical telescopes and laser-tracking stations, there are several hundreds of thousands of pieces of debris in the one- to 10-centimeter range, as well as 100 million more pieces of debris that are smaller than a centimeter, whose positions are basically unknown.
The collision scenes in the 2013 movie Gravity gruesomely illustrate what would happen if a large piece of junk were to collide with, for instance, the International Space Station. NASA reports that over the past 20 years the station has had to perform about one evasive maneuver a year to avoid space debris that is flying too close, and the trend is increasing, with three maneuvers made in 2020. Space junk has the power to significantly disturb our current way of life, which, often unbeknownst to us, largely relies on space technologies. Satellites are necessary for cell phones, television and the Internet, of course, but also global positioning, banking, Earth observations for weather predictions, emergency responses to natural disasters, transport and many other activities that are critical to our daily lives.
A number of projects are aiming to clean up space, but these efforts are technologically difficult, politically complex and expensive. Meanwhile some scientists, including our team at the Australian National University, are working to develop mitigation strategies from the ground. Working from Earth is easier and more affordable and can rely on technologies that we already do well, such as adaptive optics.
Various subtle differences exist between the way we use adaptive optics for astronomy and the way we apply it to space situational awareness. The speed of satellites depends on their distance from Earth. At the altitude of 400 kilometers above the ground, the International Space Station, for instance, is flying at the incredible pace of eight kilometers per second and completes a full orbit every hour and a half. This is much faster than the apparent motion of the sun and stars, which take a day to circle overhead due to Earth’s rotation. Because of this speed, when telescopes track satellites, the atmospheric turbulence appears to change much more rapidly, and adaptive optics systems have to make corrections 10 to 20 times faster than if they were tracking astronomical objects. We must also point the guide star laser beam slightly ahead of the satellite to probe the atmosphere where the satellite will be a few milliseconds later.
Adaptive optics can be used to track and take images of satellites and debris in low-Earth orbit and to improve the tracking of objects in low, medium and geostationary orbits. One of the ways we track space objects is light detection and ranging, a technique more commonly known as LIDAR. We project a tracking laser (not to be confused with the guide star laser) into the sky to bounce off a satellite, and we measure the time it takes to come back to us to determine the spacecraft’s precise distance to Earth. In this case, the adaptive optics system preconditions the laser beam by intentionally distorting its light before it travels through the turbulent atmosphere. We calculate our distortions to counteract the effects of turbulence so that the laser beam is undisturbed after it exits the atmosphere.
In addition to tracking space debris, we hope to be able to use this technique to push objects off course if they are heading for a collision. The small amount of pressure exerted when a photon of laser light reflects from the surface of debris could modify the orbit of an object with a large area-to-mass ratio. To be effective, we need adaptive optics to focus the laser beam precisely where we want it to go. This strategy would not reduce the amount of debris in orbit, but it could help prevent debris-on-debris collisions and possibly delay the onset of the catastrophic Kessler scenario. Eventually such systems could be employed around the globe to help manage the space environment.
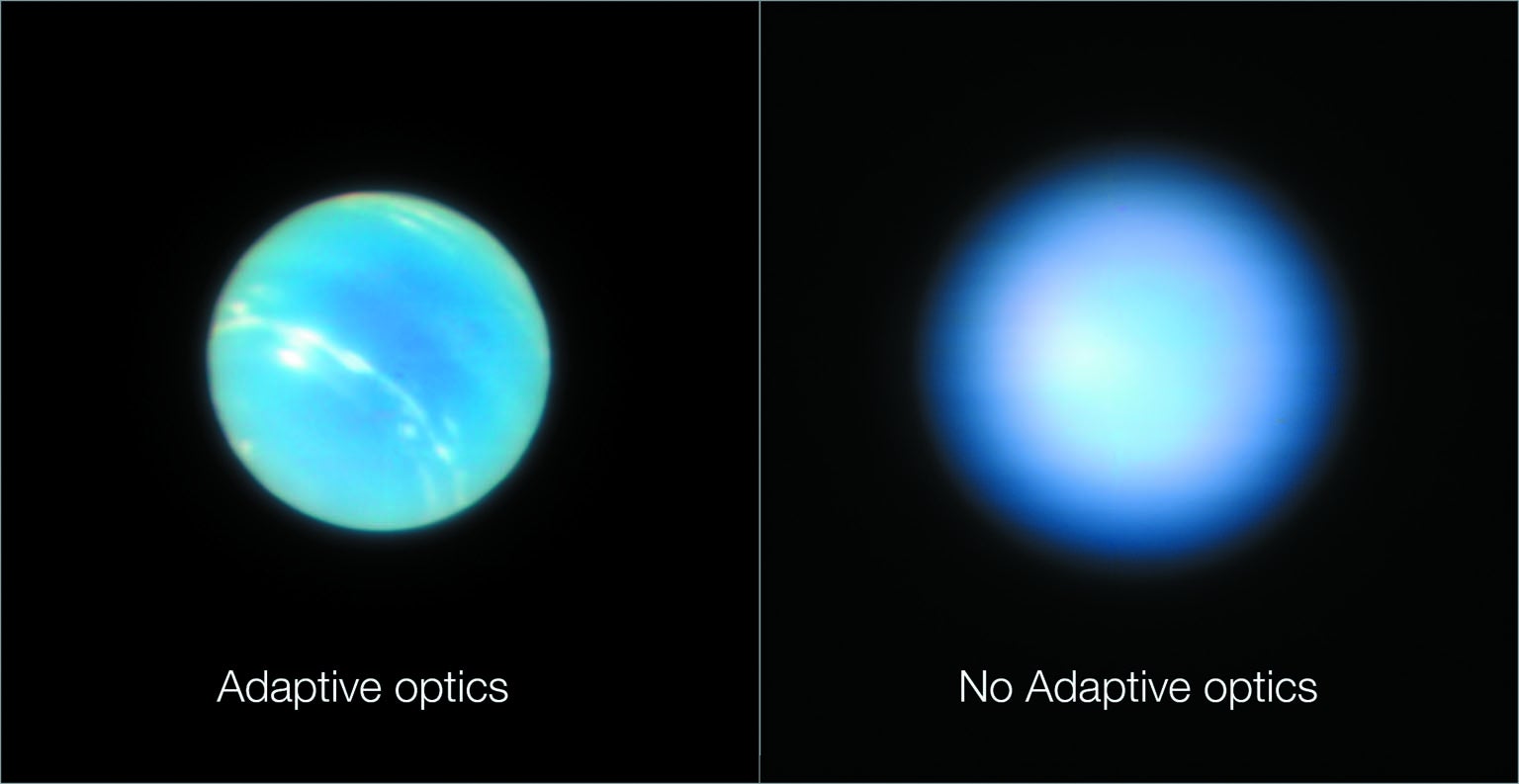
Quantum Transmissions
Space safety is not the only application that can benefit from adaptive optics. Encrypted communications are essential to many of the technological advances we have seen in recent decades. Tap-and-go payment systems from mobile phones and wristwatches, online banking and e-commerce all rely on high-speed secure communications technology. The encryption we use for these communications is based on hard-to-solve mathematical problems, and it works only because current computers cannot solve these problems fast enough to break the encryption. Quantum computers, which may soon have the ability to solve these problems faster than their classical counterparts, threaten traditional encryption. Cryptographers are constantly inventing new techniques to secure data, but no one has been able to achieve a completely secure encryption protocol. Quantum cryptography aims to change that.
Quantum encryption relies on the nature of light and the laws of quantum physics. The backbone of any quantum-encryption system is a quantum “key.” Quantum sources can provide an endless supply of truly random numbers to create keys that are unbreakable, replacing classically derived keys that are made in a predictable and therefore decipherable way. These keys can be generated at a very high rate, and we need to use them only once, thereby providing a provably unbreakable cypher.
To send a quantum-encrypted communication over long distances without a fiber-optic connection, we must transmit laser light from an optical telescope on the ground to a receiving telescope on a satellite and back again. The problem with sending these signals is the same one we face when we use a laser to push a piece of space debris: the atmosphere changes the path of the transmission. But we can use the same adaptive optics technologies to send and receive these quantum signals, vastly increasing the amount of data we can transfer. This strategy may allow optical communication to compete with large radio-frequency satellite communication dishes, with the advantage of being quantum-compatible. There are other hurdles to implementing quantum communications—for instance, the need to store and route quantum information without disturbing the quantum state. But researchers are actively working on these challenges, and we hope to eventually create a global quantum-secure network. Adaptive optics is a critical part of working toward this dream.
The Atmospheric Highway
Suddenly a technology once reserved for studying the heavens may help us meet some of the great goals of the future—protecting the safety of space and communicating securely. These new applications will in turn push adaptive optics forward, to the benefit of astronomy as well.
Traditionally adaptive optics was only viable for large observatories where the cost was justified by big performance gains. But space monitoring and communication strongly benefit from adaptive optics even on modest apertures. We find ourselves in a situation where all these communities can help one another. Undersubscribed telescopes could find a new life once equipped with adaptive optics, and space debris monitors are hungry for more telescope access to cover as many latitudes and longitudes as possible. For future observatories, astronomers are considering adding technical requirements to their telescopes and instruments to make them compatible with other space research applications such as space situational awareness and communication. Not only does it strengthen their science case, it gives them access to new sources of funding, including private enterprises.
We are entering a multidisciplinary age where the sky is a common resource. While we are sharpening images of the sky, we are blurring the lines between all the activities that use a telescope as their primary tool. Scientists and engineers building adaptive optics systems are now broadening their collaborative circles and putting themselves in the middle of this new dynamic.
Adaptive optics is also being used more without telescopes. An important and now rather mainstream use of adaptive optics is in medical imagery and ophthalmology, to correct for the aberrations introduced by imaging through living tissues and the eye. Other uses include optimum laser focusing for industrial laser tools and even antimissile military lasers. There has never been a more exciting time to explore the potential of adaptive optics in space and on Earth.