On September 9, 2018, a robotic telescope on its routine patrol of the night sky detected what looked like a new star. Over the next few hours, the “star” grew 10 times brighter, triggering a flag by software I had written to identify unusual celestial events. It was nighttime in California, and I was asleep, but my colleagues on the other side of the world reacted quickly to the alert. Twelve hours later we had obtained enough additional data from telescopes on Earth and in space to confirm that this was the explosion of a star—a supernova—in a distant galaxy. But this was no ordinary supernova.
Tying together the evidence from different telescopes, we concluded that after shining for millions of years, the star did something surprising and mysterious: it abruptly cast off layers of gas from its surface, forming a cocoon around itself. A few days or a week later the star exploded. The debris from the blast collided with the cocoon, producing an unusually bright and short-lived flash of light. Because the explosion took place in a galaxy far away—the light took almost a billion years to reach Earth—it was too dim to be seen with the naked eye but bright enough for our observatories. Through a retrospective search of telescope data, we were even able to detect the star in the act of shedding two weeks before it exploded, when it was one one-hundredth as bright as the explosion itself.
This was just one of several recent discoveries that have shown us that stars die in surprisingly diverse ways. Sometimes, for example, the remnant of a star's core that is left over after a supernova remains active after the star has collapsed—it can launch a jet of material moving at hyperrelativistic speeds, and the jet itself can destroy the star with more energy than a normal supernova. Sometimes, in the final days to years of its life, a star blows away a significant fraction of its gas in a series of violent eruptions. These extreme deaths appear to be rare, but the fact that they happen at all tells us there is much we still do not understand about the basics of how stars live and die.
Now my colleagues and I are amassing a collection of unusual stellar endings that challenge our traditional assumptions. We are beginning to be able to ask and answer fundamental questions: Which factors determine how a star dies? Why do some stars end their lives with eruptions or violent jets, while others simply explode?
A New Star
The story of stellar birth, life and death is a tale of competing forces. Stars are formed in interstellar clouds of hydrogen gas when the force of gravity pulls part of the cloud inward strongly enough to overcome the outward push of magnetic fields and gas particles traveling at high speeds. As the cloud fragment collapses, it becomes 20 orders of magnitude denser and heats up by millions of degrees—temperatures high enough for the hydrogen atoms to collide and stick together to form helium. Fusion has begun, and a new star is born.
Like a cloud, a star is itself a battleground, with gravity pushing in and pressure from nuclear fusion pushing out. The evolution of a star depends on its temperature, which in turn depends on its mass. The heavier the star, the heavier the elements it can forge, and the faster it burns through its fuel. The lightest stars fuse hydrogen to helium and stop there—the sun is more than four billion years old and is still burning its hydrogen. Heavier stars live much shorter lives, only 10 million years or so, yet manufacture a much longer chain of elements: oxygen, carbon, neon, nitrogen, magnesium, silicon and even iron.
A star's mass also determines how it will die. Lightweight stars—those that weigh less than around eight times the mass of the sun—die relatively peacefully. After exhausting their supplies of nuclear fuel, the outer layers of these stars blow out into space, forming beautiful planetary nebulae and leaving the stars' cores exposed as white dwarfs—hot, dense objects with about half the mass of the sun that are only slightly larger than Earth.
Heavier stars, however, meet a violent end because of the enormous temperatures and pressures in their cores. Around the time they reach iron in the nuclear burning chain, conditions are so hot that things fall apart—iron atoms can start breaking into smaller pieces. The chain of fusion is cut off, and the star loses its internal pressure. Gravity takes over, and the core collapses until its constituent atoms are so close together that another opposing force steps in: the strong nuclear force. Now the core has become a neutron star, an exotic and dense state of matter made mostly of neutrons. If the star is massive enough—say, more than 20 times the mass of the sun—gravity overcomes even the strong nuclear force, and the neutron star collapses further into a black hole. Either way, some of the energy released when the core collapses pushes the outer layers of the star into space, creating an explosion so bright that for a few days it outshines the rest of the stars in the galaxy combined.
Human beings have spotted supernovae by eye for thousands of years. In 1572 a Danish astronomer named Tycho Brahe noticed a new star in the constellation Cassiopeia. It was as bright as Venus and stayed that bright for months before fading away. He wrote that he was so shocked that he doubted his own eyes. Today the aftermath of the explosion—the debris—is still visible and is known as Tycho's Supernova Remnant.
For a supernova to be bright enough to be seen by the unaided eye, it must be in the Milky Way, as Tycho's supernova was, or in one of its satellite galaxies, and this is rare. I might not see a supernova without the help of a telescope in my lifetime, although I can hope. In the past century astronomers began using telescopes to find supernovae beyond the Milky Way by taking repeated observations of the same set of galaxies and looking for changes, called transients. Our telescopes are now roboticized and outfitted with modern cameras, enabling us to discover thousands of supernovae every year.
An early sign that some stars die in extreme ways was the 1960s discovery of gamma-ray bursts (GRBs), so named because of the bright blasts of gamma-ray light they emit. We believe we see them when a massive star collapses into a neutron star or a black hole, the newborn compact object launches a narrow jet of matter, that jet successfully tunnels from the core through what remains of the star, and the jet just happens to be pointing at Earth.
What might create such a jet? The basic idea is the following. When a normal star runs out of fuel and dies, its core collapses into a neutron star or a black hole, and that is the end of that. In a gamma-ray burst, however, the corpse stays active. Perhaps the nascent black hole is absorbing mass from a disk of material around it, releasing energy in the process. Or maybe the newly created neutron star is rotating quickly, and a powerful magnetic field acts as a brake, releasing energy as the star slows down. Either way, this “central engine” pumps out energy that gets funneled into a jet of extremely hot plasma that tunnels from the center of the star out through the infalling material, glowing in gamma rays.
The passage of the jet through the star causes it to explode in a special supernova dubbed “Type Ic-BL,” which is 10 times more energetic than ordinary supernovae. As the jet plows into the surrounding gas and dust, it produces light all across the electromagnetic spectrum, called an afterglow. Afterglows are difficult to find because although they are 1,000 times brighter than typical supernovae, they are 100 times more fleeting, appearing and disappearing in just a few hours. The best hope for finding an afterglow is to wait for a gamma-ray burst to be discovered by a satellite and then immediately point your telescope to the reported location of the burst.
By waiting for a satellite to discover a burst, though, you limit the kinds of phenomena you can discover. A lot of things have to go right for a GRB to be produced: the jet has to be launched, make it through the star, and be pointing at you. In fact, it seems extremely unlikely for GRBs to occur: the gamma-ray photons emitted by the jet should get trapped unless the jet is moving at 99.995 percent of the speed of light. But to reach such speeds, the jet would need to somehow make it through the star without dragging along the star's matter with it. What if most jets actually do get slowed down by the star, and we see only the small fraction that make it through unscathed? In other words, perhaps gamma-ray bursts represent the rare occasions that jets escape their stars and don't slow down too much. If that were true, there would be a huge number of extreme stellar deaths out there that are totally invisible to gamma-ray satellites.
For my thesis, I set out to find afterglows without relying on a trigger from a satellite. My plan was to use the Zwicky Transient Facility, a robotic telescope at the Palomar Observatory in California, to patrol the sky for unusually fleeting, unusually bright points of light—and then react quickly. When I presented my thesis proposal in May 2018, my faculty advisers warned me that I might not find what I was looking for. They urged me to keep an open mind because new avenues of inquiry might arise. One month later that is exactly what happened. And two years later when I graduated, my thesis looked very different from what I had expected.
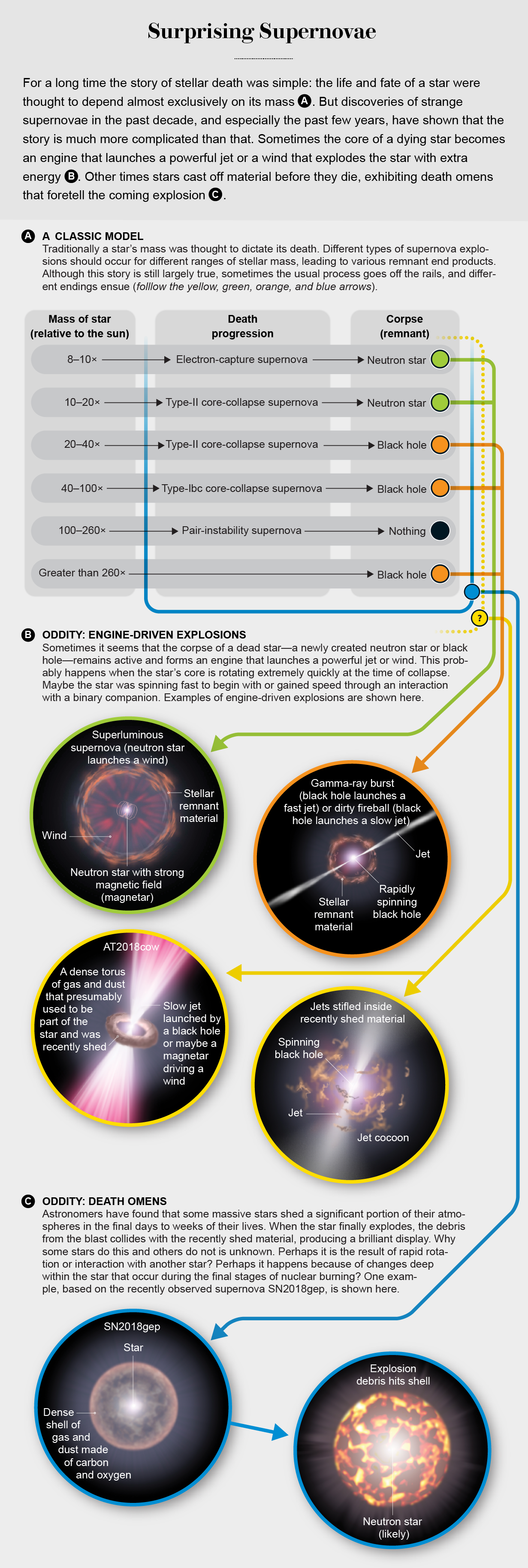
Holy Cow
When I began my work, I wrote a program to find celestial phenomena that were changing in brightness more rapidly than ordinary supernovae. On a normal day I examined 10 to 100 different candidates and concluded that none of them were what I was looking for. On some days, though, I encountered something that gave me pause.
In June 2018 I saw a report from a robotic telescope facility called ATLAS, reporting a strange event dubbed AT2018cow. “AT” stood for “astronomical transient,” the prefix automatically given to all new transients, “2018” for the year of discovery, and “cow” was a unique string of letters. In the next couple of days there were reports of similarities between this event and gamma-ray bursts, yet there had been no detected show of gamma rays. “Aha,” I thought, “this is it!” Because AT2018cow was so bright and so nearby, there was intense worldwide interest in this object, and astronomers observed it all across the electromagnetic spectrum. I immediately made plans to observe AT2018cow using a radio telescope in Hawaii called the Submillimeter Array.
AT2018cow stunned just about everyone. It unfolded completely differently than any cosmic explosion seen before. We were like the people in a classic parable who are trying to identify an elephant in the dark. One person feels its trunk and says it is a waterspout, whereas another feels the ear and thinks it must be a fan, and a third feels the leg and says it is a tree. Similarly, AT2018cow shared characteristics with several different classes of phenomena, but it has been difficult to put a complete picture together.
My collaborators and I spent long days and nights going over our data repeatedly, trying to figure out how to interpret them. Some of those moments—calculating the properties of the shock wave together on a chalkboard, a team member running down the hallway waving a piece of paper with new results, and meeting a colleague's eyes in shock when a beautiful new measurement came in—remain my most treasured memories from graduate school. In the end, we concluded that there were two important components to AT2018cow. The first was a central engine, as in a gamma-ray burst, but lasting for much longer—weeks rather than the typical days; x-rays shining from the heart of the explosion stayed bright for much longer than expected. The second was that for some reason, when the star burst apart, it was surrounded by a cocoon of gas and dust with about one one-thousandth the mass of the sun. Our evidence for the cocoon is indirect: when the star exploded, we saw a flash of optical light and radio waves that seemed to indicate debris hitting a mass surrounding the star. Such cocoons have been seen in other types of explosions, but we do not know how they get there—it may be that the material is shed by the star shortly before exploding.
If this theory is correct, it would be the first time astronomers have directly witnessed the birth of a compact object like a neutron star or a black hole; most of the time the corpse is completely shrouded by what remains of the star. In the case of AT2018cow, we think we could actually see down to the compact object that produced all of this amazingly variable and bright x-ray emission. Still, we are left with many questions. What kind of star exploded? Was the central engine a neutron star or a black hole? Why did the star shed mass shortly before exploding? To make progress, we needed to find similar events, so my colleagues and I set out to find another AT2018cow using the Zwicky Transient Facility.
Three months later I thought we found one—the bright, fast-rising explosion of September 9, 2018. Initially it looked very similar to AT2018cow. Within a week, however, it became clear that this event was a Type Ic-BL supernova—the kind associated with gamma-ray bursts. Its name was SN2018gep. I was excited. Sure, it was not another AT2018cow, but we finally had something that looked like a gamma-ray burst. Within five days we had collected detailed observations all across the electromagnetic spectrum. We searched the data for evidence of a jet—but we found none. Instead, yet again, my collaborators and I concluded that we were seeing bright, fast-evolving optical emission from the collision of explosion debris with a cocoon of material.
This was a surprise. Although cocoons have been seen surrounding other types of stars, they are not commonly observed in the types of supernovae associated with gamma-ray bursts. Our discovery implies that more stars shed gas at the end of their lives than we thought. We know the gas was lost in the final moments of the star's life because it was so close to the star at the time of the explosion; if it had been cast off earlier, it would have had time to get farther away. That means the star lost a significant chunk of its outer atmosphere in the final days to weeks of its life, after shining for millions to tens of millions of years. It seems, then, that this shedding heralds the death of the star.
Once again, we were left with questions. How prevalent are these death omens in different types of stars? What is the physical mechanism that produces them? I realized that I had a new direction to my research now—not just gamma-ray bursts and jets but also the warning signs of soon-to-explode massive stars. And perhaps these different phenomena were even connected.
It was not until the final six months of my Ph.D. program that I finally found a gamma-ray burst afterglow. On January 28, 2020, I did my usual candidate review when I saw something that looked promising. I knew better than to get excited—there had been many, many false starts over the years. I immediately requested additional observations with a telescope in La Palma in the Canary Islands, and they confirmed that this source was fading away quickly, as would be expected for an afterglow. That night I requested urgent observations on the 200-inch Hale Telescope at the Palomar Observatory that showed the source was still fading. The next night I obtained observations with the Swift X-ray space telescope and detected x-rays from the event, all but confirming this was truly a GRB afterglow. The night after that I got a brief window of time on the Keck Telescope on Mauna Kea in Hawaii, with the hope of measuring how far away the explosion was.
I slept in a sleeping bag in the remote observing room at my university, the California Institute of Technology, and set an alarm for 4 A.M. When the time came, I felt panicked—I was squeezing in this observation right at the end of the night, the sky was getting brighter quickly, the source was very faint, and I was terrified of being too late. I did the best that I could. When it was too bright to observe any longer, I called my colleague Dan Perley of Liverpool John Moores University in England on Skype, and we looked at the data together. I was lucky. The source was faint, but there was a big, booming, obvious feature in the light from the event that enabled us to measure the distance, which was vast: a redshift of 2.9, which means its light had significantly reddened during its journey through the cosmos. When this star exploded, the universe was only 2.3 billion years old. The photons from the blast took 11.4 billion years to reach Earth. Today the physical location of the burst is 21 billion light-years away—the explosion happened so long ago that the universe has expanded significantly since then. This was the real deal.
A few months after finding our first afterglow, we found a second. To put that in perspective, prior to the Zwicky Transient Facility, only three afterglows had ever been found without a gamma-ray burst first occurring and telling astronomers where to look, and we found two in just a few months. Now that we have our search strategy ironed out and working, I hope we can find these routinely. Still, even with two afterglows in hand, I cannot definitively answer the questions I originally set out to answer. It is difficult to tell whether any given afterglow is something new or just a normal gamma-ray burst that high-energy satellites happened to miss. We will need to find more events before we can tell if we are witnessing truly different phenomena.
Expanding the Catalog
Since the discovery of an unexpected new type of engine-driven explosion in AT2018cow, my search has uncovered a variety of unusual stellar displays. There was the weird Ic-BL supernova (the kind associated with GRBs) crashing into a cocoon of material but showing no evidence for a powerful jet (the hallmark of a GRB). Then there was another event similar to AT2018cow. There were also two Ic-BL supernova that probably had jets, but these were less energetic and wider than those in traditional gamma-ray bursts. And finally, right at the end of graduate school, two actual cosmological afterglows, one of which turned out to have an associated gamma-ray burst.
So far we astronomers have been like zoologists, going out into relatively uncharted territory and characterizing all the different creatures (in this case, explosions) that we see. The next stage will be to look for patterns. What are the relative rates of each type of blast? Do they seem to occur in one type of galaxy but not another? Are these different categories actually different “species” or just different manifestations of the same phenomenon?
To answer these questions, we will need a much larger catalog. Beginning in a few years, the Vera C. Rubin Observatory, currently under construction in Chile, will use the largest digital camera ever constructed (three billion pixels) to spot 10 million potential transients every night—10 times more than the Zwicky Transient Facility does now. With more data, I would like to investigate which stars lose some of their mass right before they die and how often. I want to study how we can tell if there was a jet that got choked inside a star and how to recognize the kind of faint emission emitted during a star's death throes to predict where and when a star will explode. Ultimately I would like to probe questions about the factors that lead to these unusual deaths—perhaps it is something about a star's rate of spin or its history of interactions with other stars that causes it to die in such a spectacular and rare way.