Breaking the rules is exciting, especially if they have held for a long time. This is true not just in life but also in particle physics. Here the rule I’m thinking of is called “lepton flavor universality,” and it is one of the predictions of our Standard Model of particle physics, which describes all the known fundamental particles and their interactions (except for gravity). For several decades after the invention of the Standard Model, particles seemed to obey this rule.
Things started to change in 2004, when the E821 experiment at Brookhaven National Laboratory on Long Island announced its measurement of a property of the muon—a heavy version of the electron—known as its g-factor. The measurement wasn’t what the Standard Model predicted. Muons and electrons are both part of a class of particles called leptons (along with a third particle, the tau, as well as the three generations of neutrinos). The rule of lepton flavor universality says that because electrons and muons are charged leptons, they should all interact with other particles in the same way (barring small differences related to the Higgs particle). If they don’t, then they violate lepton flavor universality—and the unexpected g-factor measurement suggested that’s just what was happening.
If particles really were breaking this rule, that would be exciting in its own right and also because physicists believe that the Standard Model can’t be the ultimate theory of nature. The theory doesn’t explain why neutrinos have mass, nor what makes up the invisible dark matter that seems to dominate the cosmos, nor why matter won out over antimatter in the early universe. Therefore, the Standard Model must be merely an approximate description that we will need to supplement by adding new particles and interactions. Physicists have proposed a huge number of such extensions, but at most one of these theories can be correct, and so far none of them has received any direct confirmation. A measured violation of the Standard Model would be a flashlight pointing the way toward this higher theory we seek.
A Trip to Elba
The E821 experiment and the discovery of mysterious muon behavior happened before my time in particle physics. I got involved in the business of lepton flavor universality violation about 10 years ago, as a postdoc in Bern, Switzerland, when I was invited to a meeting about the proposed SuperB collider to be built in Tor Vergata near Rome. The meeting was being held on the picturesque Italian island of Elba on the Tyrrhenian Sea. Though picturesque, the island is not easy to reach. The invitation was on short notice; I quickly booked a train to Pisa but missed the conference bus. Fortunately, two of the organizers offered to take me along in their car to Elba. This ride proved fortuitous.
As we drove through beautiful landscapes, we chatted about physics. One of the scientists, an experimentalist named Eugenio Paoloni, asked me what I thought about the new measurements of B meson decays by the BaBar experiment in California, which pointed toward a violation of lepton flavor universality. B mesons are particles containing a beauty quark, and they are some of physicists’ favorite particles to study because they decay in a variety of ways that have the potential to reveal new secrets of physics. I hadn’t heard about the BaBar result, probably because at the time it hadn’t attracted much attention. But I quickly thought of a possible explanation for the measurement—a new Higgs boson, in addition to the conventional one we know of, could cause the phenomena seen at BaBar. My interest in the topic of lepton flavor universality violations was born.
The rest of the workshop was uneventful. After the first day, the focus was on the development of the collider, and as a theorist I didn’t understand a word the experimentalists were saying. So I enjoyed Elba and worked on a paper about my Higgs boson idea, which I finished shortly after my return to Bern. The article got published, but unfortunately the SuperB project was canceled, and the reactions of my colleagues to the paper were not enthusiastic, to say the least: “A year from now there will be nothing left to explain by new physics” was a typical response, meaning that the measurement was probably a statistical fluke and the anomaly would disappear with more data.
For some time after the BaBar findings, there were no new results related to this question, and things grew quiet. But then, in 2013, the LHCb experiment at the Large Hadron Collider (LHC) at CERN near Geneva observed a deviation from the Standard Model prediction in a complicated quantity called P5′ (“P-five-prime”) related to how B mesons decay. On the surface, this quantity is not related to lepton flavor universality, and I didn’t find the measurement very exciting at first. My feelings changed a year later, however, when LHCb analyzed a ratio called R(K), which is a measure of lepton flavor universality violation. The experiment found a deviation from the standard expectation, and it agreed with the P5′ findings, indicating that some new phenomenon might be occurring in muons.
A little while later the story hit a turning point, again at a conference. It was once more in Italy, this time in the charming village of La Thuile in the Alps, close to Mont Blanc. During the afternoon session after the skiing break, a partial eclipse of the sun took place. Just as amazing, scientists from LHCb announced a result that confirmed the previous P5′ measurement with more statistics—and my theory friends Joaquim Matias (called Quim) and David Straub agreed on the interpretation of these data. They had never agreed before. After thanking the speakers, I said to the audience, “Today we witnessed a rare event, a partial eclipse of the sun; however, that Quim and David agree for the first time is even more remarkable.”
From then on, the evidence for lepton flavor universality violation has continued to grow. Lepton universality is an old rule, and it has been many years since we last saw a part of the Standard Model be disproved. If the rule has truly been broken, there must be new interactions and new particles in the universe that we don’t know about—potentially particles that could help solve some of the biggest mysteries of our time.
Back to Basics
To fully understand lepton flavor universality and what violating it means, we first have to review the known constituents of matter at the subatomic scale and the interactions among them—that is, the Standard Model. The building blocks of matter are called fermions, after the great physicist Enrico Fermi. These matter particles come in three versions, called generations, that are the same in every way except for their mass. For instance, the electron has heavier versions called muons and taus, the up quark has heavier relatives named charm and top quarks, and the down quark is followed by strange and beauty quarks. Only the light flavors are stable—they constitute the ordinary matter our world is made of. (Two up quarks and a down quark make a proton, and one up quark and two down quarks make a neutron.)
In addition to these particles, there are three forces through which the fermions can interact: the weak force, the strong force and the electromagnetic force (gravity is disregarded in the Standard Model because it is extremely weak at the subatomic scale). The corresponding force particles are called the W and Z bosons (for the weak force), gluons (for the strong force) and photons (for the electromagnetic force). Crucially, none of these interactions distinguishes among the three generations of fermions. The only thing that differentiates between the flavors is the famous Higgs boson, which is responsible for the fermions’ differing masses.
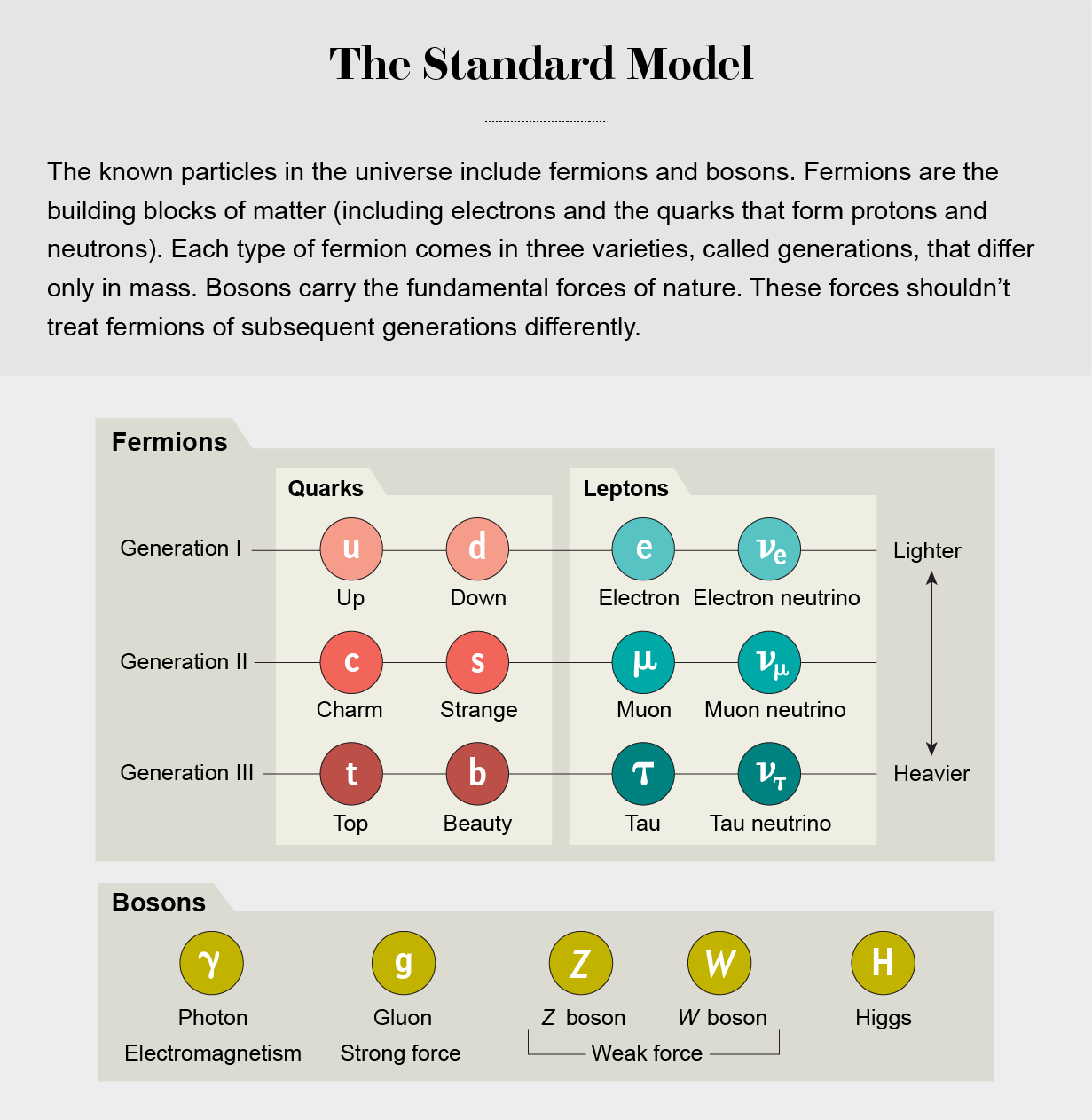
Or so we thought. If leptons are not universal—if there are forces that do discriminate among the generations—then something interesting is afoot. So far we have four different indications that lepton flavor universality might not hold true.
b → sl+l-
The first comes from measurements of a particle decay process labeled b → sl+l-, where the b represents a beauty quark, s is a strange quark and l is a charged lepton (either an electron or a muon). During this process the beauty quark turns into a strange quark and produces a pair of leptons—specifically, a lepton and its antimatter partner. We would expect these classes of decays to give rise to muons approximately as often as electrons. Yet experiments that have measured these processes, such as LHCb, observe more electrons than muons, suggesting an imbalance. The combined experimental data now indicate that there is at most a 0.0001 percent chance this difference is only a statistical fluke.
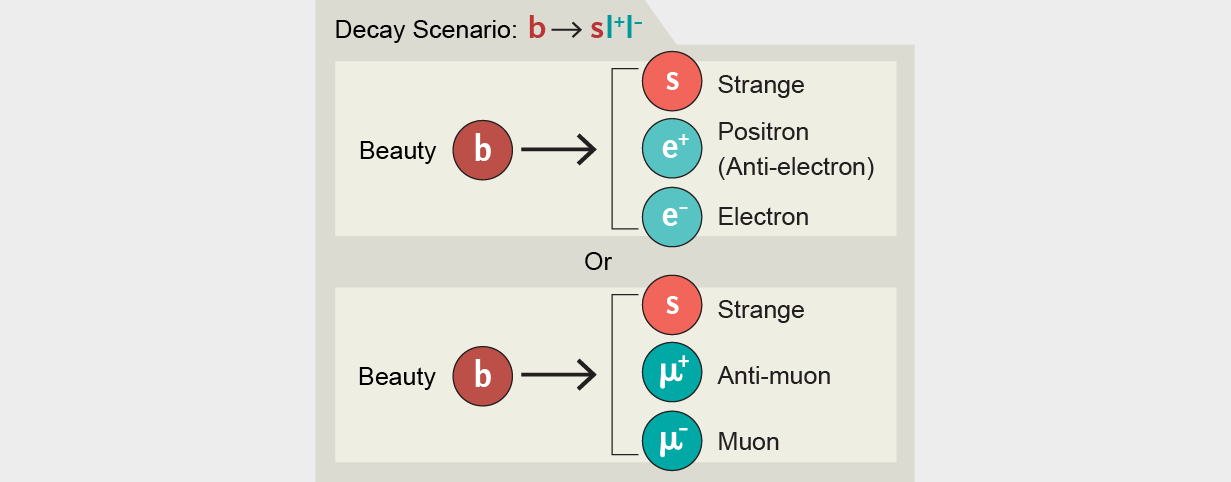
Theorists have proposed various new particles and forces that can describe the data better than the Standard Model. How, one might ask, can one account for a lack of muons by adding new particles? Explaining a deficit through an addition might seem contradictory, but this would be the case only in classical physics. In the quantum realm, it makes perfect sense. Because all particles also have wave properties, quantum mechanics predicts so-called virtual particles that appear and disappear all the time in empty space. These particles can interfere with the decay processes of regular particles, causing the decay rates to change from what the Standard Model predicts. One possibility here, for instance, is that the beauty quark, on its way to turning into its usual decay products, briefly interacts with a virtual heavy version, a new Z boson (called Z′) that, contrary to the standard Z particle, does distinguish between muons and electrons.
b → clv
The second piece of evidence for lepton universality violations comes from observing a beauty quark decaying into a charm quark (c), a lepton (l) and a neutrino (υ). Here tau leptons are expected less frequently than muons or electrons because they are heavier. Yet experiments such as BaBar, LHCb and an experiment in Japan called Belle have found that decays to tau particles happen more often than expected. Furthermore, the decays to muons and electrons show a relative asymmetry not expected in the Standard Model. Again, virtual particles may be interfering with the usual decay pathways. For instance, the beauty quark may interact with a virtual charged Higgs particle such as the one I proposed in 2012 (although this model now has some problems) or with another proposed novel particle referred to as a leptoquark.
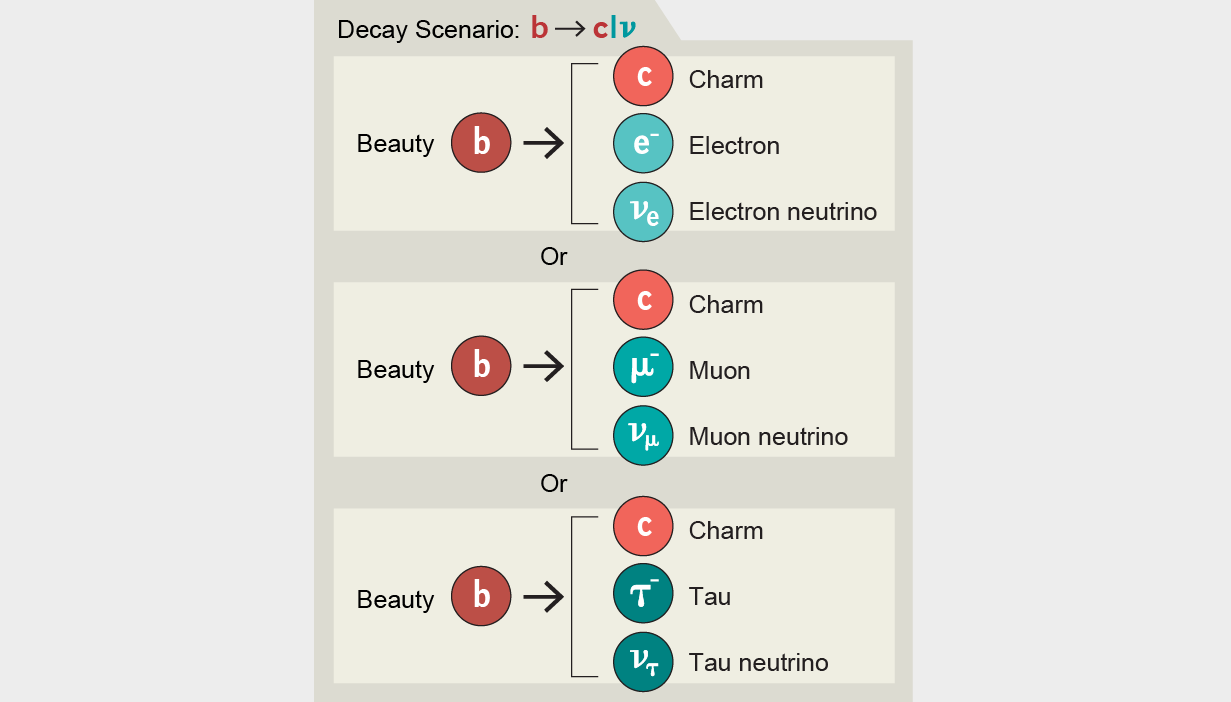
Cabibbo Angle Anomaly and qq→e+e−
Another intriguing signal comes from certain radioactive decays called nuclear beta decays. Experiments have observed that these decays happen less frequently than expected. Beta decays occur within atomic nuclei, when down quarks transform into up quarks, or vice versa, allowing a neutron to become a proton, or the reverse, by emitting an electron and an antineutrino, or a positron (the antimatter counterpart of the electron) and a neutrino. When physicists combined their measurements with improved theoretical calculations, they realized that the particles within nuclei live longer than expected. This finding, called the Cabibbo Angle Anomaly, can be interpreted as another sign that electrons and muons might behave differently.
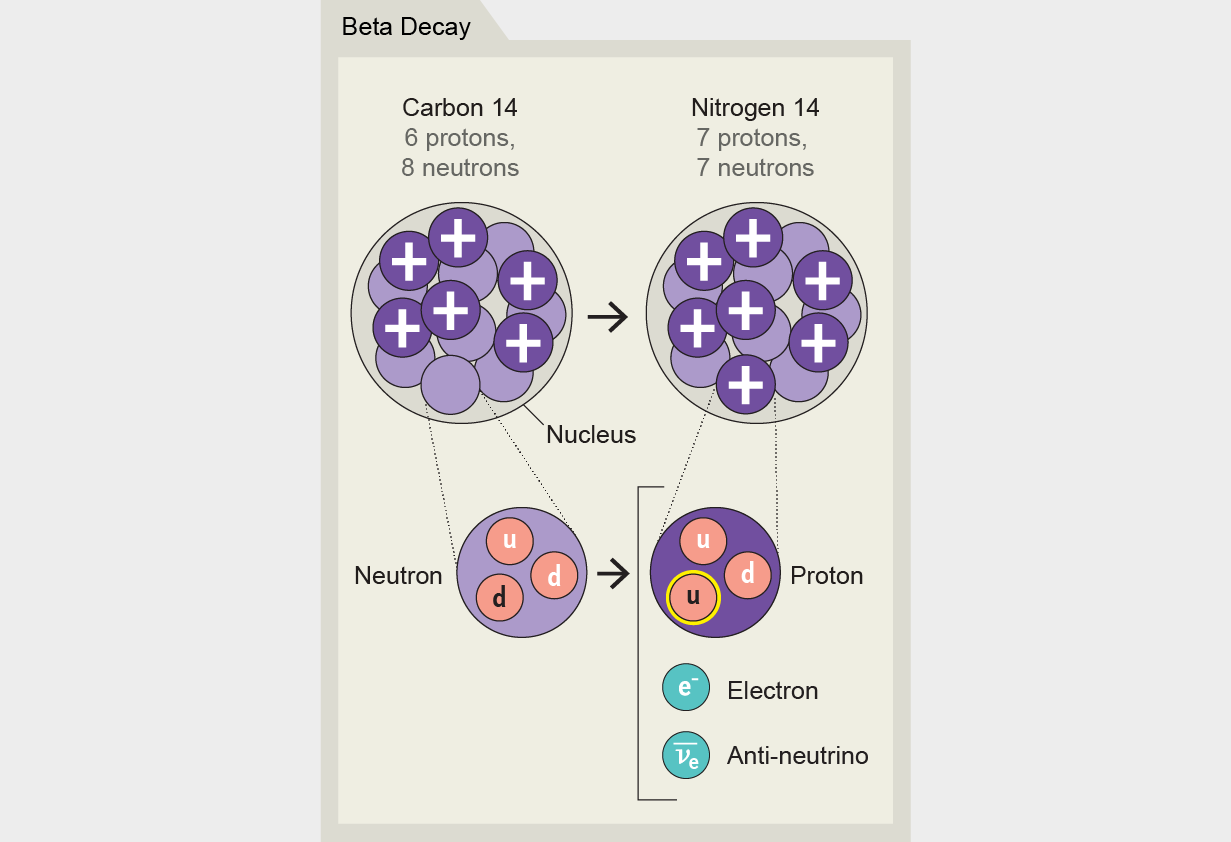
Furthermore, the CMS experiment at the LHC observed collisions of two protons that resulted in high-energy electrons (qq→e+e−) and found that more electrons were produced in comparison to muons than expected, again pointing toward the violation of lepton flavor universality. This measurement and the Cabibbo Angle Anomaly could be related because the same interaction might suppress radioactive decays but also enhance the production of high-energy electrons.
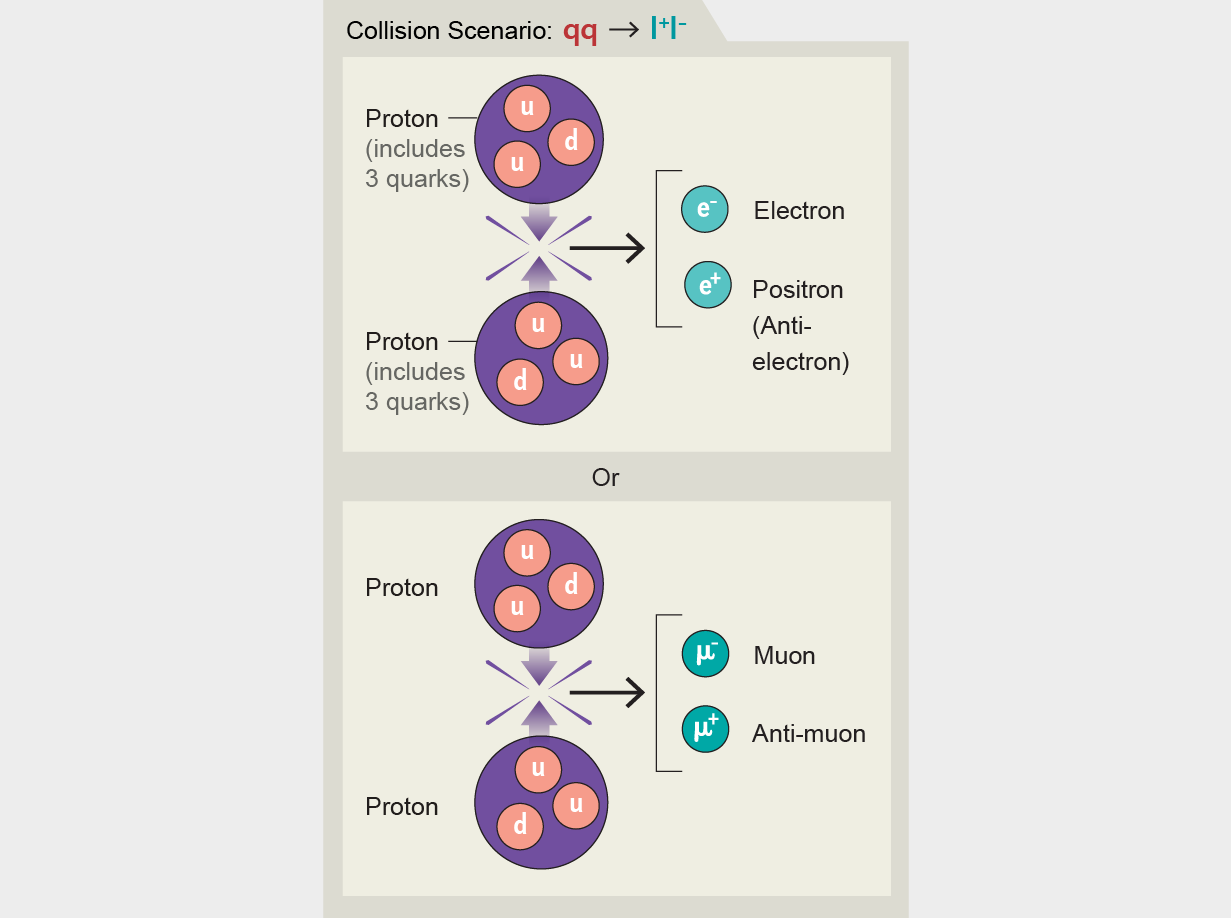
The Magnetic Moment of the Muon
This term describes how strongly a muon interacts with a magnetic field. Physicists quantify it with a g-factor, which we can predict very precisely with the Standard Model. Yet the Brookhaven experiment and the latest results coming from the G-2 experiment at Fermilab deviate from this prediction. The G-2 project sends muons around a magnetized ring and measures how their spins change as they travel. If muons were alone in the experiment, their spins would not change—but virtual particles arising around them can tug on the muons, introducing a wobble to their spins. Of course, the known particles can appear as virtual particles to cause this effect, but the Standard Model calculation accounts for that. If there are more particles in nature than the ones we are aware of, however, the experiment will see an extra wobble—and it does.
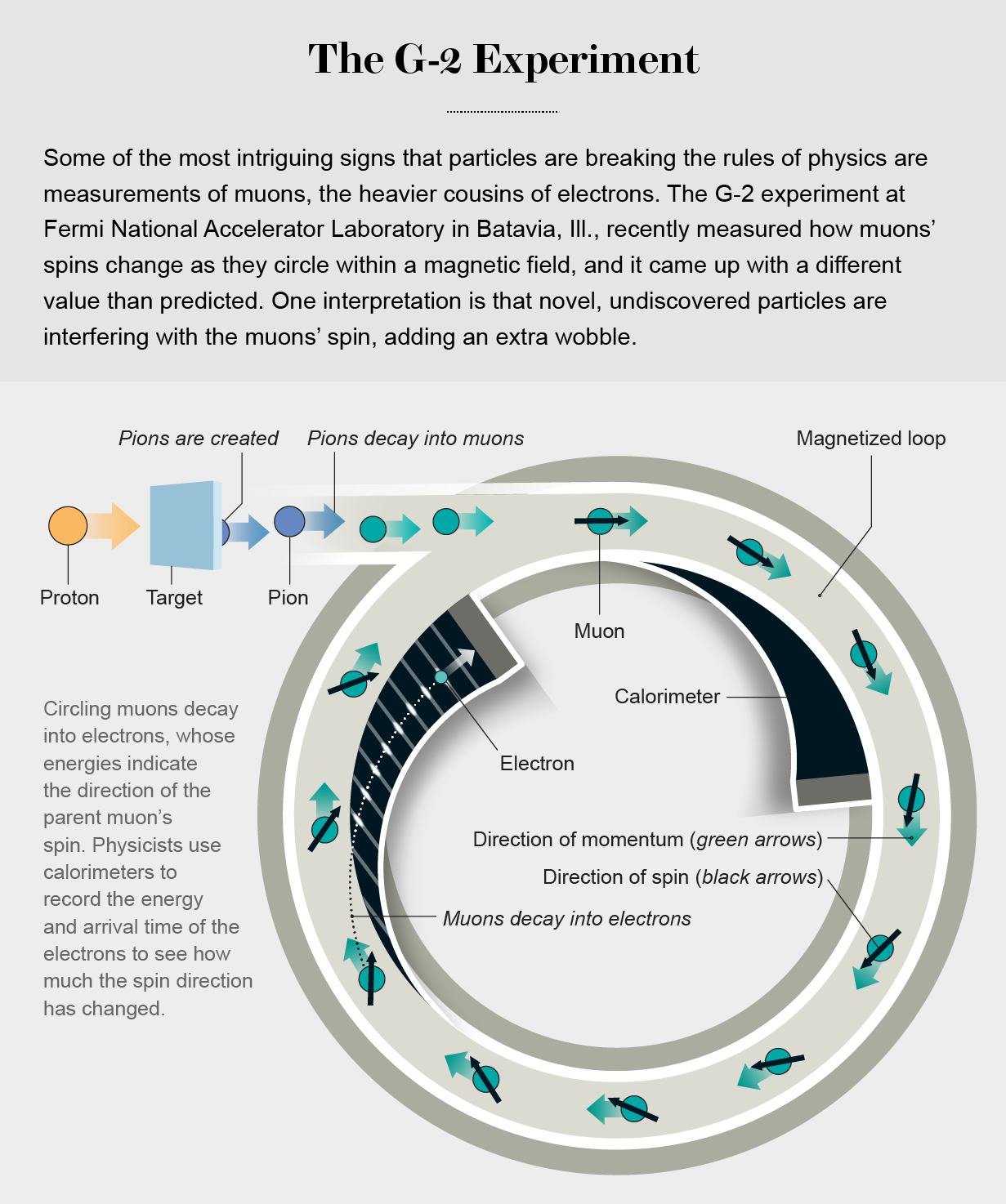
The combined results from the G-2 experiment and the previous trial at Brookhaven add up to a probability of less than 0.01 percent that this anomaly is a statistical fluke. Yet the Standard Model prediction that enables this calculation is itself questionable. It is based on other experimental results (for example, from BaBar and the KLOE project in Italy) that do not agree with simulations of quantum field theory that were recently performed on supercomputers.
A New Particle Zoo
If we must extend the Standard Model to account for these anomalies, how should we do it? In other words, how can we modify the equations describing nature so that theory and experiment agree?
Particles in one promising class that are capable of explaining these measurements are called leptoquarks. They connect a single quark directly to a single lepton: for instance, a lepton could transform into a quark by emitting a leptoquark—unlike any interaction in the Standard Model. Such a particle would be something radically new. It has been proposed in the past in the context of grand unified theories, which were devised to unite the different forces in the Standard Model at high energies. These high energies, however, would correspond to particles that are very heavy. Physicists would need to alter existing grand unified models to create a leptoquark light enough to affect the measurements we have discussed.
Another option involves other new particles, such as heavy fermions, heavy “scalar” particles (including new Higgs bosons), or novel gauge bosons (similar to the W and Z bosons). One intriguing way to predict such particles is by using theories that contain, in addition to our four dimensions (three of space and one of time), at least one extra dimension, compactly folded up and hidden within the ones we know.
Although these hints we have for new phenomena are very intriguing—at least in my view—it’s critical that we corroborate these hints with additional, more precise data and more accurate theoretical calculations. A number of experiments and theoretical collaborations worldwide are working on this challenge. These include the LHCb experiment, which started collecting new data when the LHC began its most recent run this summer. The Belle II experiment in Japan, which is dedicated to investigating B meson decays, is also gathering new evidence. If just one of these anomalies were confirmed, it would prove the existence of new particles or interactions. Furthermore, it would mean that the new particles must have masses that could be probed directly at the LHC or a future collider. These novel particles would also affect other phenomena we can observe, allowing physicists to make complementary tests of the new particles’ properties.
Future accelerators could provide further insights. An electron-positron collider, such as the Future Circular Collider (FCC-ee) planned at CERN or the Circular Electron Positron Collider (CEPC) to be built in China, should have a sufficiently high luminosity (meaning they produce enough collisions) to create large numbers of Z bosons. These are useful for observing predicted deviations from the Standard Model in several ways. First, most anomalies, in particular the anomalous magnetic moment of the muon, would affect Z decays, such as Z bosons turning into a muon and an antimatter muon. Second, the Z bosons expected at the FCC-ee would produce an unprecedented number of beauty quarks and tau leptons. Large numbers of these particles would allow for precise tests of the decay processes for which we expect to see effects from new particles—effects that are currently not detectable because we lack enough data to see a strong signal. An electron-positron collider could start operating around 2040. Later, physicists hope to collide protons in the same tunnel (the machine would then be called the FCC-hh), producing much higher energies and potentially creating the particles directly. Such a collider would probably not open before 2060, however. I would need a very healthy lifestyle to see one of the models I’ve worked on confirmed.
We are at an exciting point in this exploration. Results are constantly being updated and questioned. Very recently new theory calculations bolstered the case for new physics in b → sl+l− and b → clν decays; meanwhile, there are rumors about the reliability of the corresponding experimental measurements. We are all eagerly awaiting updated measurements and further improved theoretical predictions. If the present hints of lepton universality violation hold up, they could provide long-sought guidance toward a more complete fundamental theory of particle physics. We hope such a theory will finally resolve some of our biggest questions about nature—neutrino masses, dark matter and the missing antimatter in our universe.